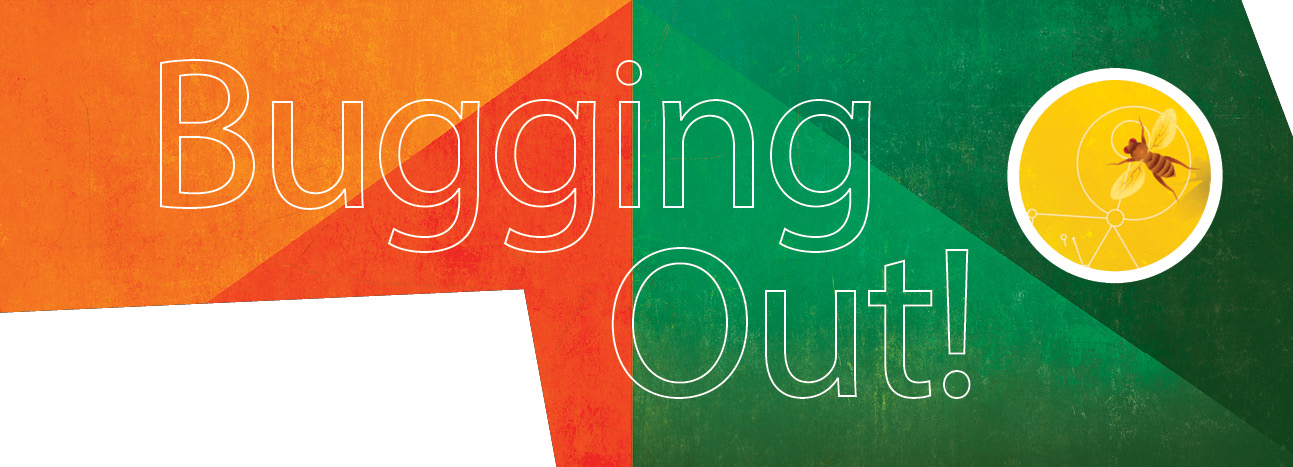
Insects and arachnids inspire crucial research across the research ecosystem at Johns Hopkins—from robot design to tumor tracking.
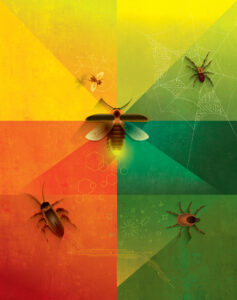
From the classic 1950s science fiction film Them! to last year’s Jurassic World Dominion, bugs have long been portrayed as threats to humankind’s existence. It’s easy to see why: There are more than 10 quintillion insects and arachnids on Earth—1.4 billion of them for every one of us. Let that sink in!
The truth is, life as we know it wouldn’t exist without bees, flies, spiders, ticks, beetles, and the more than 900,000 other bug species that share our planet. Without their work pollinating crops, serving as food for birds and other animals, and breaking down and recycling soil nutrients, our ecosystem would simply collapse.
But these creatures also play a crucial role in the research ecosystem at Johns Hopkins, from inspiring the design of robots and other mechanical systems to lending their unique chemistry to medical diagnostics and treatments. Here we spotlight a few of these projects.
Lighting the Way to Better Medical Treatments
by Lisa Ercolano
Fireflies belong to the summer, floating above lush green lawns like a glowing galaxy come to Earth to illuminate warm evenings.
But in a laboratory on Johns Hopkins’ East Baltimore campus, the winged beetles—or at least, the enzyme that makes them glimmer and shine—are lighting the way to better treatment for everything from cancer to tissue damaged by amyotrophic lateral sclerosis, also known as Lou Gehrig’s disease.
“We use stem cells to treat and understand a number of ailments, and it’s important for us to be able to track and follow these cells once they are in the body. Luciferase, the enzyme that makes fireflies glow, lets us do that,” says Ana Rosu, a senior biomedical engineering major working in the lab of Jeff Bulte, an affiliate in the Johns Hopkins Institute for NanoBioTechology and faculty member at the Johns Hopkins University School of Medicine.
Fireflies (also called “lightning bugs”) use luciferase, another chemical called luciferin, oxygen, and ATP (energy-carrying molecules found in all cells) to kindle light in specialized organs located in their abdomens so they can communicate with each other and find mates.
Rosu and Bulte’s team leverages that chemical reaction by injecting stem cells that contain luciferase into animal models and following up with a chaser of luciferin, causing the original cells to illuminate. Then the researchers use sensitive optical imaging instruments to follow these cells, beaming like infinitesimal car headlights navigating a dark roadway, on their journey throughout the body.
“Because only live cells can oxidize luciferin and produce light, we can use the light imaging signal as a surrogate marker for cell survival to answer the two important questions: how long our injected cells survive, and when [targeted] tumor cells are starting to die,” Rosu explains.
This is crucial information in the team’s investigation into Lou Gehrig’s and other diseases that cause damage to the myelin: the protective sheath surrounding nerve fibers in the brain, eyes, and spinal cord.
“Sometimes, stem cells are rejected by the host, so we need to give immunosuppressants. But it is important to optimize treatment protocols so the stem cells that are there as therapy can survive as long as they can. So we use the luciferase chemical reaction on various days, allowing us to see how long the therapeutic stem cells survive,” Rosu says.
The team is also using stem cells to deliver gold and bismuth nanoparticles to tumors, which can kill the cancerous cells. Once the cells arrive, the researchers apply a laser to heat up the particles, “frying the tumor,” Rosu notes.
“So what we do is engineer the tumor cells with luciferase before we implant them, and then we inject luciferin daily afterwards to see how many cancer cells—if any—survive,” she says.
Move Over, Charlotte: There’s Another Spider in Town
by Lisa Ercolano
Mechanical engineering doctoral student Eugene Lin has a confession: Spiders used to make him shiver, especially big, hairy, scurrying ones.
But these days, one of Lin’s closest companions is a 10-inch, thick-legged robotic spider that crouches on a web fashioned from parachute cord, waiting patiently for humans to activate the controller that will bring it to life.
This cyborg arachnid, modeled on Uloborus diversus— an orb-weaver common to the U.S.—may hold the key to understanding the complex vibrational system spiders use to sense and capture their prey: knowledge that could one day enable the design of machines, such as autonomous vehicles, with similar sensing abilities.
“The first step is understanding the spider’s sensing. This kind of spider is essentially blind, so how does it locate and grab its prey? That little robot up there is helping us find out,” says Lin, who is part of the Terradynamics Lab team in Hackerman Hall, which is led by Chen Li, assistant professor of mechanical engineering.
The team designed and built the mechanical spider from the ground up, informed by a trove of data gleaned from the lab of collaborator Andrew Gordus, an assistant professor of biology in the Krieger School of Arts and Sciences. Gordus studies the behavior, neural circuits, and genetics of Uloborus diversus in hundreds of living specimens that are raised and reproduce in the campus greenhouse.
So why build a robot model of a spider when you could just walk across the quad and observe the real thing?
Lin explains that researchers like using robots as active physical models of organisms for many reasons, including that models can be manipulated and controlled in ways that living creatures cannot, enabling researchers to test various hypotheses. The models also allow researchers to measure many facets of a system simultaneously, which can be challenging in real biological systems. In addition, models are simpler and easier to study to discover general principles, he says.
In the case of Lin’s spider, the team not only can adjust the posture of the robot and the tension of the web, but also can record and analyze the robot’s movements with two highspeed cameras that extract 3D coordinates of each location on the web to quantify how it is vibrating.
“What we’ve learned so far is that the behavior of the robotic spider and its robotic prey [a vibrating magnet clipped to a thread of the web] influences how vibrations propagate through the web and the legs of the robotic spider, but we still have a long way to go,” Lin says.
First up may be adding a few more legs to the model. Right now it has only four.
“Yeah, that’s next on our list,” Lin says.
Cockroaches and Their Model Behavior
by various contributors
Encountered in pantries and kitchens, cockroaches are despised as ugly, unhealthy pests: something to be eliminated with a spritz of pesticide or the quick crush of a shoe heel. In the Terradynamics Lab on the Homewood campus, however, they are part of a research program aimed at developing robots that can traverse rough terrain as nimbly as the much-maligned insects can.
“In the wild, cockroaches are tenacious and can easily navigate dense vegetation and very complex terrain. If they tip over, they flip themselves back on their feet and just keep on going,” says Chen Li, assistant professor of mechanical engineering and Terradynamics Lab director. “We are trying to understand how they do this, and then apply those principles to create advanced robots who can do the same.”
Cockroach-inspired robots could, for instance, be deployed into post-earthquake rubble to perform search-and-rescue missions or explore the alien surface of another planet. To that end, Li’s team uses cameras to closely track the movements of the bugs (in this case, Blaberus discoidalis, also known as the discoid cockroach because of its shape) as they traverse various terrains, from tracks featuring gaps and bumps to obstacle courses studded with tall, bendable plates mounted on springs that are designed to mimic blades of grass.
In a recent study, Li’s team tracked how the insects transitioned between two types of movements—pushing, when the roaches shoved their bodies against the blades, so the blades gave way, and rolling, when the roaches spun into gaps between the blades to slide through. The researchers then digitally reconstructed the insects’ and blades’ 3D motion to examine how both movements looked on a landscape (which they call a “map”) showing how the potential energy of both the creatures and blades influenced how the roaches’ bodies moved.
“This landscape describes the combined effect of gravity and the beams’ elastic bending forces acting on the animal body, just like a gravity field or electric field can describe forces acting on a point mass or charge,” says Yaqing Wang, a doctoral student working with Li on roaches. “A key difference is that the roach is self-propelled by its legs, generating thrust, so instead of moving passively like a point mass or charge, it can actively propel itself on the potential energy landscape to traverse obstacles.”
The team learned that transitioning from pushing to rolling is a move from one potential energy “basin” to another, requiring the roach to summon enough energy to overcome an energy barrier. They also discovered that the same potential energy landscape approach applies to understanding how roaches traverse other types of obstacles, and even to how they get back on their feet after flipping over.
Li says this new energy landscape approach clarifies how animals use physical interaction to transition between different types of movements and will assist in the design of robots that can better traverse complex 3D terrain like earthquake rubble and cluttered rocks on the moon or on Mars.
Tracking Tick Bites
by Gina Wadas
The woods are lovely, dark, and deep, but can harbor a menace: a tiny insect whose bite sickens almost half a million Americans a year. Lyme disease causes a range of symptoms that include fever, rash, and joint pain, as well as effects on the central nervous system and heart. Though it’s common knowledge that Borrelia burgdorferi—the bacteria that causes the disease—enters the body through the bite of an infected deer tick, how the bacteria migrate from that bite into a person’s bloodstream has not been clearly understood.
Led by Joseph R. and Lynn C. Reynolds Professor of Materials Science Peter Searson, a team of Johns Hopkins researchers may have found the answer. Using a customdesigned 3D tissue-engineered model, they learned that B. burgdorferi uses tenacious trial-and-error movements to find and slip through tiny openings called junctions in the lining of blood vessels near the original bite site. This allows them to hitch a ride on the bloodstream throughout the body, potentially infecting other tissues and organs. Their results appeared in Advanced Science.
“Our observations showed that if the bacteria did not find one of these junctions on the first try, they continued searching until one was found,” says Searson, a core researcher at Johns Hopkins Institute for NanoBioTechnology. “The bacteria spend an hour or two using this behavior to find their way into the blood vessels, but once there, they are in circulation in a matter of seconds.”
The team injected its 3D model, which simulates a human blood vessel and its surrounding dermal tissue, with the bacteria, simulating a tick bite, and used a high-resolution optical imaging technique to observe its movements. They observed that though the tissue at the original bite site was an obstacle for the spiral-shaped bacteria to surmount, little effort was needed for them to penetrate the junctions and enter the bloodstream.
Lyme disease is prevalent in North America, Europe, and Asia, and though antibiotic treatments are effective, some patients experience symptoms that can persist for months, and, in some cases, years. The team says that understanding how B. burgdorferi spreads throughout the body could help inform treatments to prevent bacteria from the initial bite entering other tissues and organs.
“We also believe that the kind of human tissue-engineered model we created can be broadly applied to visualize the details of dynamic processes associated with other vector-borne diseases and not just Lyme disease,” Searson says.
Flying High: A First for Brain Research
by Jill Rosen
In the 1600s, French philosopher René Descartes said “I think, therefore I am.” Almost four centuries later, how we think remains largely a mystery.
Can the brain of a baby fruit fly shed some light on that process?
A team of researchers from Johns Hopkins University and the University of Cambridge believes so. They recently completed a map of that infinitesimal organ—smaller than a poppy seed—a landmark achievement in neuroscience that brings scientists closer to a true understanding of the mechanism of thought.
The map is a detailed diagram tracing every neural connection in the brain of the larval insect, an archetypal scientific model with brains comparable to that of humans.
The work, likely to underpin future brain research and to inspire new machine learning architectures, appeared in Science.
“If we want to understand who we are and how we think, part of that is understanding the mechanism of thought,” says senior author Joshua Vogelstein, an assistant professor of biomedical engineering who specializes in data-driven projects including connectomics, the study of nervous system connections. “And the key to that is knowing how neurons connect with each other.”
The first attempt at mapping a brain—a 14-year study of the roundworm begun in the 1970s—resulted in a partial map and a Nobel Prize. Since then, partial connectomes have been mapped in many systems, including flies, mice, and even humans, but these reconstructions typically represent only a tiny fraction of the total brain.
Comprehensive connectomes have only been generated for several small species with a few hundred to a few thousand neurons in their bodies—a roundworm, a larval sea squirt, and a larval marine annelid worm.
This team’s connectome of Drosophila melanogaster larva is the most complete as well as the most expansive map of an entire insect brain ever completed. It includes 3,016 neurons and every connection between them: 548,000.
“It’s been 50 years, and this is the first brain connectome. It’s a flag in the sand that we can do this,” Vogelstein says. “Everything has been working up to this.”
The team chose the fruit fly larva because the species shares much of its fundamental biology with humans, including a comparable genetic foundation. It also has rich learning and decision-making behaviors, making it a useful model organism in neuroscience. And for practical purposes, its relatively compact brain can be imaged and its circuits reconstructed within a reasonable time frame.
Even so, the work took the University of Cambridge and Johns Hopkins 12 years. The imaging alone took about a day per neuron.
In the end, the full team charted every neuron and every connection and categorized each neuron by the role it plays in the brain. They found that the brain’s busiest circuits were those that led to and away from neurons of the learning center.
The methods Johns Hopkins developed are applicable to any brain connection project, and their code is available to whomever attempts to map an even larger animal brain, Vogelstein says.
“What we learned about code for fruit flies will have implications for the code for humans,” Vogelstein says. “That’s what we want to understand—how to write a program that leads to a human brain network.”