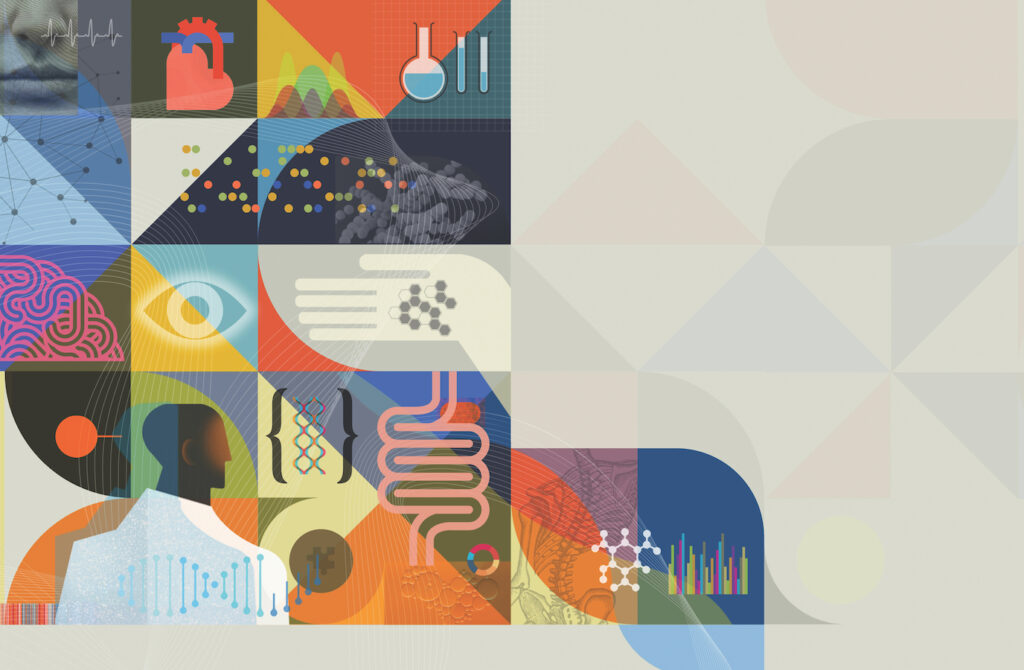
Imagine if after a serious accident, your damaged facial bones could be replaced with tissue made by your own cells. Or if you could pop a pill that could reprogram your immune system to fight a chronic disease, freeing you from a lifetime of medications. While both prospects sound futuristic, scientists and engineers at Johns Hopkins and elsewhere are working toward these and other advances in tissue engineering.
Some researchers are creating novel biomaterials that can be transplanted into the body to serve as replacement parts or to encourage new tissue growth. Other scientists are developing small replicas of human tissues or organs that not only can be used to study the impact of novel drugs or treatments but also be encouraged to communicate with replicas of different organ systems. Still others are focused on using a new avenue known as immunoengineering to reprogram the immune system to tolerate organ and tissue transplants and medical devices, and using regenerative immunotherapies to direct tissue reconstruction.
“Some ideas always seem like science fiction before they become science fact,” says Joshua Doloff, an assistant professor of biomedical engineering and of materials science and engineering. “That’s just to help increase imagination and creative thinking, and eventually you tackle a really difficult problem.”
In the roundup that follows, we highlight some of the most promising projects now underway at Johns Hopkins.
Jump to a Section
- Bone and Facial Reconstruction
- Cellular-Level Technologies
- Soft Tissue
- Heart
- Peripheral Nerves
- Vasculature
- Pancreas
- Harnessing the Immune System
Bone and Facial Reconstruction
Patients experiencing craniofacial bone loss resulting from trauma or cancer removal surgeries have a few options today for reconstructive surgeries. But they all have limitations. Autografts, a process that involves taking a piece of bone from another body part to transplant to the face, create a second defect. Allografts (which use donor tissue) hold the potential for disease transfer or rejection. Surgeons also can employ materials like metals to serve as an implant; these, too, are subject to possible rejection and don’t move like native tissue.
Enter Warren Grayson. The professor of biomedical engineering has been leading efforts to regenerate bonelike tissue with natural anatomical structure: living tissue that grows and changes with the patient and is made from a patient’s own genetic material so it won’t be rejected.
In studies in mice and pigs, Grayson’s lab has used 3D printers to create scaffolds made from biodegradable polymers combined with other natural materials in the exact shape of the facial defects. Then, they take stem cells derived from fat tissue and apply them to the scaffold in a liquid solution. The idea is the cells will grow throughout the porous scaffold, which provides instructive cues so the cells form bone. The scaffold then degrades, leaving new, vibrant bone tissue.
Over the years, the Grayson lab has tinkered with various scaffold materials, methods to stimulate stem cells, and recipes for optimal bone growth. Animal studies have demonstrated that some bone can be regrown in this manner. “There’s definitely room for improvement,” Grayson says, “but the results have been extremely promising. The data tell you that you can induce the body to go beyond its normal healing capacity.”
In related work, Grayson and colleagues are applying similar processes to regenerate skeletal muscle and are developing new imaging tools to better study how these materials work once transplanted into the body. Grayson’s group is applying for funding to conduct pilot clinical studies in humans. How quickly things move from there will depend on results and larger-scale clinical trials.
- On the Horizon: Growing replacement facial bones in the lab for transplant to humans
- Timetable: 3 to 5 years, for clinical studies
Cellular-Level Technologies
The human body holds trillions of cells that function like tiny computers, processing inputs that direct them to grow, divide, specialize, or self-destruct, explains Jordan Green, professor of biomedical engineering.
Green’s lab has taken a cue from viruses — which trick cells into letting them enter and then use this space to replicate — to develop novel biodegradable nanobiomaterials that can deliver medications or genetic material in miniature packages (1/1,000th the size of a human hair) directly to specific types of cells, thereby reprogramming them from the inside out.
The process could be used to deliver DNA and RNA to cells to turn genes on or off individually or in combination. This holds potential for common genetic conditions, such as cystic fibrosis or hemophilia, as well as more complicated diseases, like cancer or diabetes, Green says. It even can help hit targets traditionally seen by pharmaceutical companies as “undruggable.”
Green and colleagues also are working on developing different sizes and shapes of artificial immune cells to mimic biological cells, with proteins added to the surface that teach native immune cells what to do, such as attack cancers or promote tissue repair. A recent study demonstrated that lab-developed nanoparticles using molecules called poly(beta-amino ester)s, along with an engineered sequence of DNA, could reprogram liver cancer cells to secrete a protein called TRAIL that initiates cancer cell death. Combining this approach with small-molecule drugs could offer a new potential approach for cancer treatment.
“The wave of the future is doing in situ tissue engineering using administered gene therapy and immunotherapy to promote healing and regeneration of tissues within the body,” Green says. “We’re going to see these technologies more and more.”
- On the Horizon: Gene therapies, stem cell therapies, and immunotherapies that can deliver treatments, promote tolerance to foreign bodies, and promote healing
- Timetable: 5 years for gene therapies and vaccines; 5 to 10 years for stem cell therapies
Soft Tissue
People who have lost soft tissue, whether as the result of a congenital disease or due to trauma or cancer surgery, present a challenge to surgeons. Doctors can transplant fat tissue harvested from elsewhere in the body, but without supporting blood vessel networks, the transplanted fat tends to die over time. Some patients don’t have enough soft tissue to spare for the transplant, and synthetic materials, such as silicon gels used in breast implants, can induce foreign-body reactions by the immune system.
Ideally, says Hai-Quan Mao, professor of materials science and engineering and of biomedical engineering, “You want the material to be porous enough to allow host tissue to grow into it but also retain its shape and the integrity of the repair site, and not lead to scarring and fibrosis by host immune cells. This concept is easy to understand, but it was difficult to come up with a material design to meet all of those requirements simultaneously.”
Mao’s team, working with Johns Hopkins plastic and reconstructive surgeons Sashank Reddy and Justin Sacks (now at Washington University in St. Louis), has invented a new type of synthetic soft tissue substitute that is well-tolerated and encourages the growth of soft tissue and blood vessels.
As a model, researchers observed the microscopic structure of fat, consisting of large cells clustered around an extracellular matrix that provides shape and stability. To devise a new biomaterial, they used a hydrogel made of hyaluronic acid — a naturally occurring component of the body’s extracellular matrix and an ingredient found in more than 90% of cosmetic dermal fillers used in this country. Then, they incorporated nanofibers of polycaprolactone, the same material used in some resorbable sutures, to help the material retain its shape. They broke the fibers into short segments before making the composite gel to ensure easy injection through a needle. The resulting composite gel performed well in several animal studies, repairing deformities while encouraging growth of new blood vessels.
Mao and his co-investigators founded a company called LifeSprout that is conducting phase 1 clinical trials of small amounts (1 to 3 cubic centimeters) of the soft tissue substitute in the face for removing wrinkles or adding volume to a facial structure, before developing a strategy for larger-volume repairs.
They have been awarded a Maryland Stem Cell Research Fund grant to test this material for larger-volume repair, to see if they can speed and enhance regeneration by adding fat tissue-derived stem cells.
- On the Horizon: Injecting soft tissue material to fill in defects and combining donor stem cells from a bank or stem cells taken from a patient’s fat tissue with synthetic soft tissue material for repair
- Timetable: 5 to 6 years, to allow time for regulatory approval
Heart
Deok-Ho Kim’s research in cardiac tissue engineering is out of this world. Kim, an associate professor of biomedical engineering, has developed 3D engineered cardiac tissues that mimic the microarchitecture and function of human heart tissue on a microchip.
The work made news headlines in March 2020, when Kim and colleagues sent some of their miniaturized heart tissues with SpaceX to the International Space Station for a month to study how space travel and weightlessness — which has been known to simulate accelerated aging — affects the heart’s structure and function. Kim’s team observed that contractile force and heart function declined compared to similar tissues studied on Earth.
Sponsored by the National Institutes of Health and NASA, the team now is gearing up for a second space launch in fall 2022. This time, the engineered heart tissue chips will be treated with some type of drug compound or mechanical stimulation (muscle conditioning) to see if diminishing function can be prevented or lessened. The lab has been testing various interventions using a desktop-sized random positioning machine that simulates microgravity. A companion project funded by NASA is focused on monitoring how radiation from the sun and other elements in space impacts changes in human cardiac tissues.
In other work, Kim, David Kass (professor of medicine and biomedical engineering), and their colleagues are validating 3D engineered muscular tissues as a “clinical trial on a chip” platform to study cardiac and skeletal muscle deficiencies in human Duchenne and Becker muscular dystrophies, inherited conditions marked by progressive muscle weakness. By placing these patients’ induced pluripotent stem cell-derived muscular tissues in multiwell plates in the lab, investigators will simulate clinical trials of a novel drug for muscular dystrophy, assessing toxicity profiles, helping determine appropriate doses, and potentially personalizing therapy for patients. This avoids typical problems experienced with clinical trials, such as patient recruitment, patient dropout, and harmful side effects.
A platform of heart and skeletal muscle tissues to test new drugs already is commercially available through Curi Bio, a company Kim co-founded in 2015.
In separate studies, Leslie Tung, professor of biomedical engineering, is using engineered heart slices to study electrophysiological conditions related to heart disease, such as arrhythmogenic cardiomyopathy, a cause of sudden cardiac death. His team seeds human induced pluripotent stem cells from patients onto a scaffold of slices of heart tissue from rats or pigs that have been stripped of their native cells. This serves as a model system to study heart arrhythmias and their potential treatments in the lab.
- On the Horizon: New treatments or therapies to help protect astronauts’ health on long missions, treat age-related heart diseases, or electrophysiology conditions
- Timetable: To be determined
Peripheral Nerves
A couple of years ago, Johns Hopkins plastic and reconstructive surgeon Sami Tuffaha approached Hai-Quan Mao with a vexing problem.
Tuffaha is an expert on targeted muscle reinnervation, a peripheral nerve repair procedure for amputees during which each severed nerve at the amputation site is sutured to a smaller motor nerve in a neighboring muscle. This helps prevent the disorganized growth of nerve stumps into painful tumors called neuromas. However, the size difference between the two nerve stumps being connected creates additional technical challenges and still leads to neuroma development in some 30% of patients.
Three engineering undergraduate students stepped up to find a solution. Under Mao’s direction, Michael Lan ’21 (BME), Bruce Enzmann (MatSci) and Anson Zhou (BME) devised a flexible, cone-shaped device as a conduit to guide proper nerve growth over the different-sized nerves.
Similar to a simple funnel, the biodegradable device has an outer porous, extendable, cone-shaped synthetic polymeric conduit filled with a biodegradable gel. The team created the conduit by spinning a polymer solution into a nanofiber mesh under a high-voltage electrical field. The mesh was then molded into a cone shape. The ends of the conduit can easily be sutured to the two nerve stumps. The students filled the inside of the conduit with a biodegradable hydrogel that included a nerve inhibitor to reduce nerve growth and shrink nerve size as it grows through the conduit. Together, these components guide and regulate proper connections between the two nerve stumps.
The COVID-19 pandemic didn’t slow down this team. With a new member, third-year student Juan Diego Carrizo (MatSci), they continued to work together via Zoom and prepared the prototype devices in a temporary space set up by the team. In pilot studies in rats — conducted by medical student Erica Lee in the Tuffaha group — the team observed the device achieved a tapered shape of regrown nerve within the device. It also prevented neuroma formation and generated fewer pain fibers compared to the TMR procedure itself.
The team has filed a provisional patent on its work under a spinoff company named Innerva. With a few funding sources — including $10,000 from the 2021 national Lemelson-MIT Student Prize competition for collegiate inventors and $85,000 from the Johns Hopkins University Cohen Translational Engineering Fund — the team is continuing to refine the device.
- On the Horizon: Lan, Enzmann, Zhou, and Carrizo will be working with Johns Hopkins Technology Ventures to work through their next steps toward additional preclinical and clinical testing and commercializing the device.
- Timetable: An estimated 5 to 7 years until clinical use
Vasculature
Feilim Mac Gabhann likes to ask his students to name the human body’s most important organ. The usual suspects emerge: heart, brain, occasionally skin. But it’s a trick question. In Mac Gabhann’s eyes, it’s blood.
“If we didn’t have blood, we’d be 1 millimeter in size,” says Mac Gabhann, associate professor of biomedical engineering. “The only reason we can be large, multiorgan organisms is because we have some sort of system that can move oxygen around the body to communicate among different organs.”
Most people are familiar with the blood vessels they can see in their arms or their feet, he says. These vessels, like interstate highways, carry blood to smaller and smaller branches supporting all tissues in the body. Any given piece of tissue is packed with networks of tiny blood vessels, 1,000 times thinner than those we can observe.
Currently, some larger vessels that become injured or diseased, such as in the leg or heart, can be surgically repaired or bolstered, or replaced with synthetic materials. Patches of damaged skin can be repaired through skin grafts. Growing large amounts of tissue or thicker tissues, however, would be “a major next step,” Mac Gabhann says, and requires creating the right environment to encourage blood vessel growth.
Mac Gabhann’s lab has been using computational modeling — the application of computers to simulate and study complex systems — to study vascular endothelial growth factor, a protein family heavily involved in blood vessel growth and development. The lab’s members want to know why and how these proteins direct the growth of varying blood vessel networks supporting different areas of the body. “If we can understand that,” he says, “we can turn it into a forward engineering problem and design the signals we need to get the kind of networks we want.”
He’s looking primarily at two areas. One is skeletal muscle, working to develop a medical intervention that could help people with peripheral artery disease, a form of blockages of blood vessels in the leg that make it painful to exercise. The other is developmental biology, attempting to understand how blood vessel networks behave in growing tissues.
- On the Horizon: Increasing the size and complexity of tissues that can be derived from stem cells; creating 3D organs from a person’s own cells or from donor cells that are compatible or that could be altered through gene editing tools
- Timetable: 10 years
Pancreas
Joshua Doloff’s lab explores the intersection between therapeutics and living systems, with a goal of understanding what happens when new materials are introduced into the body and how the host immune system behaves and perceives them. An implanted glucose monitor functioning as an artificial pancreas for those with diabetes, for example, can be attacked by the body as foreign. This can lead to the development of scar tissue around the device, diminishing its function.
Implanting devices can create a one-two punch for the immune system, Doloff notes. Doctors want to deliver grafts correctly to restore function, but if they’re implanted in the midst of a chaotic autoimmune disorder, two immune challenges emerge: preventing graft rejection and modulating the immune system to prevent further damage. He’s also pursuing immunoengineering studies to help prevent rejection of grafts.
In recent work, Doloff’s team has demonstrated that loading implantable devices with a crystallized immunosuppressant drug that is slowly released at the local area can inhibit the immune response against those devices. The research team (not the lab) is also working on ways to encapsulate islet cells and transplant them into patients with diabetes, potentially to replace nonfunctioning pancreatic cells and eliminate the necessity for insulin injections or long-term immunosuppressant drugs needed with implanted devices and organ transplants.
“Over the past few years, we’ve gotten a lot of insights into foreign-body response,” he says. “We can actually block biomaterial-specific responses quite effectively. And I’m confident that we can do that now for not just biomaterials but even multicomponent devices, such as glucose monitors.”
- On the Horizon: Medical implants that aren’t rejected by the natural immune system
- Timetable: 5 to 10 years
Harnessing the Immune System
The launch of the Translational Tissue Engineering Center at Johns Hopkins in 2010 laid the foundation for many of today’s advances. Directed by biomedical engineering professor Jennifer Elisseeff, a pioneer in regenerative immunology, the center was founded as a joint venture between the Wilmer Eye Institute and the Department of Biomedical Engineering. In 2019, the university created the Johns Hopkins Translational ImmunoEngineering Biotechnology Research Center with a $6.7 million grant from the National Institutes of Health.