In the time it takes to read this sentence, 50,000 cells in your body will die and be replaced with new cells. Lined up end to end, the body’s more than 10 billion nerve cells would stretch 45 miles. Each day, you breathe an average of 23,040 times, and your blood makes its daily 60,000-mile trip through your body. The laundry list of what’s contained in every square inch of human skin is just as mind-boggling: four yards of nerve fibers, 1,300 nerve cells, 100 sweat glands, three million cells, and three yards of blood vessels. It’s all part of what is arguably the most complex engineering system on Earth: the human body.
From the intricacies and mysteries of brain function to the speed at which a sneeze leaves your mouth—it can be in excess of 100 miles per hour—the human body is an engineering marvel. Even more so, when you consider that outside of reproductive organs, the components are basically the same in every model, but every one of the more than 6.5 billion models on the planet is genetically unique.
For all its sophistication and wonder, the human body is also a system that is prone to disease, decay, and injury. Organ failure, cancer, spinal cord injuries, blood clots, and amputations: Every day, these issues and more affect millions of people. With cutting-edge research in tissue and cell engineering and neural prosthetics, Whiting School faculty and students are helping to change the way we think about the human body—and how we heal it.
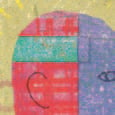
Brain
Noah Cowan, assistant professor of mechanical engineering, spends his time thinking about things most of us would rather not. Cockroaches scurrying along a wall. The eerie glow of the transparent electric knifefish. His discoveries about how the brains of these lowly creatures direct movement could eventually lead to advances in rehabilitative therapies for stroke patients, people with cerebral palsy and other debilitating conditions, and better, less jerky prosthetics. “The interface between brain and machine is only partially understood,” says Cowan. “Our results may one day be used to guide the design of brain-controlled prosthetic limbs so that they match our own natural performance.”
Cowan’s Locomotion in Mechanical & Biological Systems (LIMBS) Laboratory has spent the past four years studying the way the cockroach uses thousands of antennae sensors to navigate in the dark and dash across rooms. The cockroach’s brain processes sensory information as quickly as the bug speeds along a wall at a remarkable 25 body lengths per second. LIMBS researchers found that the curve of the antennae against a wall “reads” the shape of the wall, signaling the cockroach how fast to turn.
Using their data, Cowan and his students built an orange-and-black wheeled metal “cockroach” the size of a shoebox, complete with a flexible, rubber-like antenna. Several flex sensors measure how much the antenna bends as it moves against a wall. A computer program translates the information into distance data, which the robot, just like its six-legged inspiration, uses to judge and adjust how far it is from a wall. In addition to medical applications, their research, which landed on the May 2006 cover of The Journal of Experimental Biology, may help in developing better robotic navigation in collapsed buildings, caves, and various emergency situations.
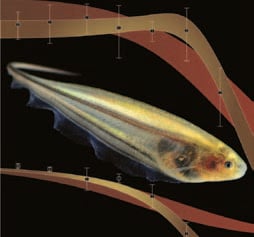
Thanks to the electricity emitted from the small, South American knifefish, Cowan and Eric Fortune, assistant professor of psychological and brain sciences in the Krieger School of Arts and Sciences, are shedding new light on the complexities of human locomotion. Though the two professors are next-door neighbors in north Baltimore, neither knew the other’s area of expertise until 2004 when Cowan asked Fortune’s daughter what her father did at Hopkins. “She told me that he poked fish brains, and my eyes lit up,” says Cowan. “Eric wasn’t applying mechanical analysis, but doing cutting-edge neurobiology with the fish [to study the neural basis and evolution of behavior]. It was like peanut butter and chocolate. Neither of us could have done this alone.”
Just four hours after Cowan first visited Fortune’s lab, the duo had the pilot data for their first successful grant from the National Science Foundation. “The knifefish’s ribbon fin can generate a force that moves it forward and backward in a line,” Cowan explains of their experiments in which they place the fish in an open-ended tube and move the tube back and forth. “The fish swims its fins off to stay in the tube.”
Using its eyes and sensory cues from the weak electric signal the nocturnal fish uses to “see” in the dark, the fish instinctively measures velocity and makes the necessary speed adjustments to remain in the tube. The team’s engineering analyses demonstrate, for the first time, that Newton’s laws of motion are hard-wired in the fish’s brain—a discovery that has broader implications for all animals. “By examining the sensory processing systems of a diversity of animals, we begin to see that every animal has to move mass through space and make continual adjustments using sensory feedback from all over the body,” Cowan says of their discovery, which was published in the January 31, 2007, issue of The Journal of Neuroscience. “Understanding how the brain processes this overwhelming amount of information is crucial if we want to help people overcome pathologies.”
To dream big, professor Andreas Andreou ’86 thinks small. Very, very small. Two years ago, he and his research team in the Whiting School’s Department of Electrical and Computer Engineering (ECE) began work on a silicon cortex using nanoscale 3-D silicon on insulator complementary metal oxide semiconductor (SOI-CMOS) technology. Roughly 4,000 times thinner than the human-hair-width 2-D microchips used in today’s computers, this new technology allows Andreou to stack multiple chips to enable the design of highly interconnected circuitry that comes closer to the brain’s neural hardware than ever before. The silicon cortex chip could potentially be used to overcome brain injuries and degeneration and to augment electronic interfaces to advanced prostheses.
“The brain isn’t a super computer,” explains Andreou, who received his PhD in 1986 from Hopkins before joining the Whiting School faculty almost two decades ago. “It’s an incredible network with the computing occurring directly in the network. One of the challenges in engineering silicon brains is that the cortex has enormous connectivity that, until recently, we couldn’t replicate in silicon.” Using SOI-CMOS technology to create a silicon retina and cochlea, combined with his research in speech and language processing (he co-founded the school’s Center for Speech and Language Processing with retired ECE professor Moise Goldstein), inspired Andreou’s next leap. “The 3-D silicon technology allows us to go beyond the limits that appear on the horizon of technology,” he says. “Now we can think about making a silicon brain that will have true cognitive abilities.”
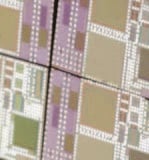
The way we perceive the world is through our eyes, ears, and skin. If we could augment cognition with a silicon cortex, why not? There are a few times I wish I had more brain power,” he says.
After testing and tinkering with their first prototype, Andreou’s team synthesized the second generation 3-D silicon cortex chip, which is currently being manufactured. “Like babies, these chips come out in nine months,” he jokes.
The 3-D silicon cortex chip complements other research in his lab. Electrical and computer engineering PhD student Miriam Alderstein Marwick has used CMOS technology to design a silicon photoreceptor that can detect single photons and hence can see in the dark, just like rods in the retina. According to Andreou, its performance is comparable and even exceeds that of biological systems.
“When I started my career, we said that we’d never be able to make a silicon brain,” notes Andreou, who in 2005 was named an Institute of Electrical and Electronics Engineers (IEEE) Fellow for his groundbreaking work in energy efficient sensory microsystems. “I was scared at first when we started developing the silicon cortex, but what is humanity? We need to have an open mind.”
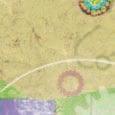
Molecules/Cells
“We have the technology. We can make him better than he was. Better… stronger… faster.” Marc Ostermeier can recite verbatim the iconic catchphrase from Six Million Dollar Man, the 1970s TV show he watched as a kid about an astronaut-cum-cyborg-cum-government operative.
Today, as an associate professor of chemical and biomolecular engineering at the Whiting School, Ostermeier doesn’t engineer high-tech arms, legs, eyes, or ears like the scientists on the TV show. Instead, he focuses on the fundamentals.
“Proteins are the molecule that nature chose to carry out the widest variety of functions,” Ostermeier explains. Some of the body’s thousands of proteins act as switches, enabling cells to respond to complex signals. In 2004, he and his research team discovered how to successfully join two unrelated proteins to engineer a protein switch, which can be cued to turn on and off.
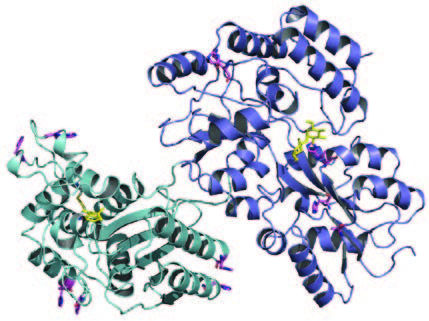
The therapeutic possibilities of repeatable cell-to-cell communication are far-reaching. One example is chemotherapy, which eradicates cancer cells—but also poisons healthy cells. “By engineering a protein to be a switch that would bond to a chemo drug and only recognize something on a cancer cell as its release trigger, chemotherapy could be selectively administered to cancer cells only,” says Ostermeier. “This is the kind of application we are shooting for.” Disease detection is another desired end product of the research in the Ostermeier lab. His team is working on creating a switch that would light up fluorescently when it senses certain cellular activity, such as certain molecules indicative of cancer.
Now Ostermeier’s challenge is to make the switches better…stronger. With funding from the National Institutes of Health, National Science Foundation, and the JHU Institute for NanoBioTechnology, his team is perfecting a model to duplicate the complex way nature improves proteins. “How life works in the end comes down to the molecular level,” he says. “Protein switches are a large part of the reason why we’re more than the sum of our parts.
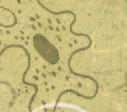
Collagen
Supermodels and Hollywood starlets aren’t the only ones who use collagen. “If you look at any animal, collagen is the basic framework for any tissue,” says Michael Yu, assistant professor in Materials Science and Engineering. “Almost 30 percent of our body’s protein is collagen.” This common, non-toxic protein is the workhorse of the human body. It promotes blood clotting, rarely triggers rejection, and its sponge-like scaffolding is essential for cells to grow nerves, bones, and skin. Collagen is also the framework for Yu’s research.
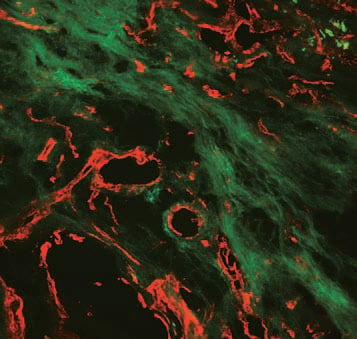
In August 2005, he pioneered a new, easy way to modify collagen by mixing it with minute molecules called collagen mimetic peptides. Prior to this, collagen was altered by cooking it at a high heat or by mixing it with intense chemicals, techniques that can damage the protein itself, as well as cells and other bioactive molecules that co-exist with collagen. Yu’s technique attaches the “hitch-hiking” mimetic peptide to collagen, binding it to the collagen’s triple-helix structure. Like any good marriage, the tiny peptide fills in gaps in the collagen’s rope-like configuration. The peptide is loyal, too, attaching only to the collagen.
In February, Yu received a National Science Foundation Career Award to perfect his technique (see p. 5) and to create biomedical applications for modified collagen, such as using it to thwart the formation of scar tissue. Modified collagen may also help to prevent organ transplant rejection by ramping up blood vessel vascularization—the speed of the process and the proliferation of blood vessels during vascularization are critical to successful transplants. In addition, blood clots in arteries and tumors could be detected through imaging techniques using the collagen mimetic peptides, and a collagen-based bandage could fight infection longer and more effectively.
He says the ultimate goal, though, is to engineer tissue and create new organs. “Recently we’ve been exploring how our technique could be used to make artificial corneas. If all medical tools fail, the last alternative is to replace an organ. My research begins where medicine’s options typically end.”
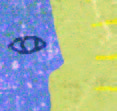
Eyes
Consider the cornea. As the clear part of the eye’s outer wall, it covers and protects the iris and pupil. As the eye’s camera, it transmits light and refracts images onto the retina, enabling us to see the world around us. Damaged corneas from disease, injury, or infection are the fourth leading cause of blindness in the world. Each year in the United States, an estimated 30,000 corneal transplants are conducted, making the surgery—which involves replacing a disc-shaped segment of the cornea with a similarly shaped piece from a healthy, donated cornea—by far the most common of all transplant operations today.
Consider the drawbacks, though. Corneal transplants are rejected 5 to 30 percent of the time, and the operation can lead to infection, bleeding, and glaucoma. Then there’s the constant need for donated corneal tissue.
Jennifer Elisseeff, assistant professor of biomedical engineering, considers this and more as she engineers artificial adhesives for the eye to repair corneal damage and repair and seal the incisions made during cataract procedures. The research team in her Biomaterials and Tissue Engineering Lab at the Whitaker Biomedical Engineering Institute at Johns Hopkins works closely with Oliver Schein, the Burton E. Grossman Professor of Ophthalmology at Hopkins Wilmer Eye Institute. Eventually, their collaborative research may lead to artificial corneas that could be used in transplants.
Three years ago, Schein approached Elisseeff about partnering to pioneer materials for the eye to be used in corneal and cataract surgery. “It’s a new field,” says Elisseeff of their biomaterials, which have just begun animal trials. “The chemistry is still very synthetic for materials used in the eye today, such as contacts. There is strong potential for incorporating [many] more biological materials into the eye. We try to do novel, innovative strategies.”
Elisseeff uses hydrogel scaffolds—a 3-D network for cells to rebuild tissue—to engineer artificial corneal tissue that mimics the way real corneal cells divide, differentiate, and proliferate. “Growing cells is an art,” she says. “The engineering part is important. You’re tinkering and building tissues that can integrate with the body.”
Limbs
Open. Shut. Open. Shut. The shiny, blue robotic replacement hand after Darth Vader whacks off the real one with the whoosh of his light saber fist. An undergraduate student wearing a bonnet-like cap fitted with non-invasive electrodes sits in front of the hand. As he thinks about moving his hand, a computer captures the change in his brainwaves and opens and closes the mechanical hand on command.
Other robotic hands rest on nearby lab tables, their palms and fingers as graceful and smooth as those of a Greek statue. Unlike their sci-fi counterpart flexing its fingers a few feet away, these hands look human with skin-like cosmesis. The 20-plus student and faculty research team in Nitish Thakor’s Biomedical Instrumentation and Neuroengineering Lab at the Johns Hopkins School of Medicine are working hard to make sure these state-of-theart prosthetics function as human hands, too.
The hands “feel” temperature through sensors embedded in the fingertips of the cosmesis. The sensors heat up or cool down depending on the temperature of the object it touches. And thanks to flexible force sensors mounted on the prosthesis, and a shoulder harness providing vibrating haptic and vibrotactile feedback, the hand can also sense how much pressure to apply when picking up a pencil or paper cup or squeezing a ketchup bottle.
The robotic hands under development represent the next generation of prosthetics: neurally controlled devices that recall brain signals and decode and interpret them in real time. These prototypes are light-years beyond the standard prosthetic hand available today: the Otto Bock hand, a “Captain Hook-like” device that opens and shuts through EMG electrodes that sit on the skin above an amputee’s muscles. “Most amputees today don’t use their prosthetic limb on a daily basis because the limb is bulky, mechanically heavy, and not aesthetically pleasing,” explains Soumyadipta Acharya, a PhD candidate in Biomedical Engineering and part of Thakor’s research team. “It’s a grand engineering project to make a limb that is a huge advance over the current prosthetic. This project is revolutionizing the way we control and feel artificial limbs using signals from our brain, nerves, and muscles directly.”
It’s also helping to kick-start a whole new field, something Thakor knows a thing or two about. Twenty years ago, Thakor, who holds his tenure and conducts research through the Johns Hopkins School of Medicine and teaches in the Whiting School of Engineering, began his Hopkins career working with the implantable defibrillator, or pacemaker, a now-standard cardiac device that was first developed and implanted in a patient at Hopkins. “Now the exciting new frontier is neuro and rehabilitation engineering” exclaims Thakor. “The idea is to bring technology to solve problems in an innovative manner and to advance science in application for problems in the brain sciences and neurobiological diseases and disorders. We are devising methods to diagnose brain disorders and injuries and developing implantable brain stimulators using brain signals to control prosthetic limbs. These are new territories and an exciting future awaits us.”
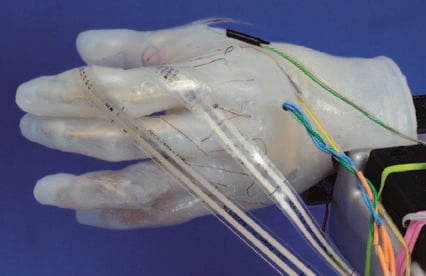
In June 2006, his team performed the first, successful demonstration of a brain-controlled prosthetic hand moving individual fingers. Their research is part of Revolutionizing Prosthetics 2009, a program sponsored by the Defense Advanced Research Projects Agency (DARPA) to create a neurally controlled upperlimb prosthetic ready for FDA approval and clinical trials by 2009. Last year, DARPA awarded Hopkins’ Applied Physics Lab (APL) $30.4 million as part of an international consortium of more than 10 organizations committed to bringing better prosthetics to market for people with limb loss as a result of accidents, disease, birth defects, and war.
Thakor oversees several sub-projects of the APL grant including: skin-like cosmesis; sensors for touch, force and temperature; prosthetic EEG control; and decoding neurons to control individual fingers and dexterous motions of the next-generation prosthetic hand. The Whiting School’s Ralph Etienne-Cummings, an associate professor in the Department of Electrical and Computer Engineering, consults on the central pattern generator (specialized neural circuits that produce rhythmic outputs to control motor systems), and Allison Okamura, an associate professor in Mechanical Engineering, provides the expertise on haptic feedback.
The Revolutionizing Prosthetics 2009 Program, managed by Stuart Harshbarger at APL, is testing its first limb system. Known as Proto 1, it was used in early clinical investigations with partners at the Rehabilitation Institute of Chicago. It is a significant step forward in naturally-controlled limbs and the perception of sensory interactions.
This summer, Thakor and his team will begin testing their prototypes with amputees. Vikram Aggarwal, a master’s student in Biomedical Engineering, finds it all a little humbling. “When you’re working, you can get lost in the details of the nitty-gritty of the neurons,” he remarks. “But in the end, this is not just advancing science, it’s improving someone’s quality of life.”
Thakor agrees. “While the challenges of interfacing to the nervous system and decoding its message are huge, the potential for making an impact is equally great. We have the knowledge and the technology is within our reach; we now have to make it work for the benefit of the amputees.”
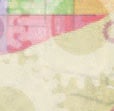
Joints
Jennifer Elisseeff knows what it’s like to walk the halls of a hospital and listen to patients complain about stiff joints and the chronic pain that often accompanies arthritis and other cartilage defects. After all, she spent two years in medical school at Harvard University while pursuing her PhD in biomedical engineering from the Harvard– MIT Division of Health Sciences and Technology.
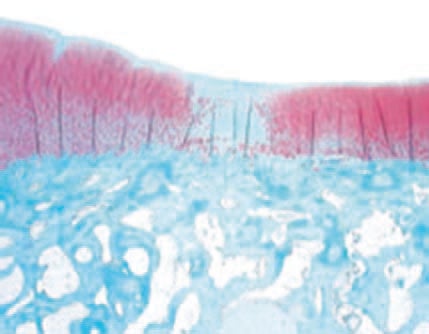
In her Biomaterials and Tissue Engineering Lab in the Johns Hopkins Whitaker Biomedical Engineering Institute, she’s developing artificial cartilage for clinical applications, focusing on both form and function. Elisseeff is designing and manipulating a sophisticated synthetic scaffold to create cartilage that will bond better to the body’s natural cartilage and soft tissue. She notes that the current biomaterials used as artificial cartilage have a tendency to not stay in place. “Our research is a combination of applied and basic research,” she explains. “We look at how bone and cartilage talk with each other. They’ve always communicated with each other, but science hasn’t understood it.”
To create a better bonding cartilage, she examines adult and embryonic stem cells to see how they rebuild and form tissue. By creating multi-layered hydrogels to serve as scaffolds, Elisseeff and her team are able to mimic the complex, 3-D networks of musculoskeletal tissue— research that will lead directly to a better understanding of cell behavior and tissue regeneration. This first for the biomaterials field was published in Nature Materials in April.
All of this, she says, is leading to a groundbreaking end-goal: a minimally invasive, injectable chondral implant to be used mostly with knees. “It will be used for treating cartilage defects that can lead to arthritis later in life and as an arthritis treatment. Patients want to avoid metal and plastic implants. We’re trying to repair tissue with the same natural material that was lost.”
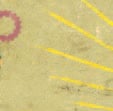
Spinal Cord
In the Department of Electrical and Computer Engineering, Associate Professor Ralph Etienne-Cummings finds inspiration on the path less taken. As a pioneer in the field of neuromorphicengineering, he designs biologically inspired robotics that walk, run, and strut with grace and agility—very much a step in a different direction from the field’s standard of using strict mathematical and control theory to produce less natural robotic movements. “Biological systems have evolved over millions of years and hence should offer very efficient examples of what engineered systems can do,” says Etienne-Cummings. “No computer can remotely perform tasks routinely performed by some of the simplest living organisms.”
Etienne-Cummings’ research in sensorymotor integration has led him to examine such humble organisms as the lamprey eel and the housefly. Currently, the common cat is helping his Computational Sensory Motor Systems Lab create new pathways—and new hope—for the treatment of spinal cord injuries. In 2006, his lab, in collaboration with Vivian Mushahwar, of the Department of Biomedical Engineering and Center for Neuroscience at the University of Alberta, Edmondson in Canada, conducted the first-ever, successful experiment with an artificial central pattern generator (CPG) that replicates a neural circuit in the spinal cord in vivo.
To demonstrate that the silicon version could replace the biological version, they implanted electrodes in a paralyzed cat’s leg muscles. Sensors attached to the cat’s legs fed input to the CPG, which is no larger than a pencil eraser and was designed by Francesco Tenore, a Whiting School PhD student in Electrical Engineering. When activated, the CPG controlled the leg muscles, and the cat walked along a three-meter-long platform. Data reveals that the CPG-generated motor activation patterns are comparable to those produced by its biological counterpart.
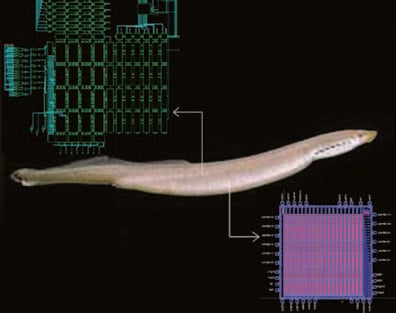
For the cat, it may have been small steps. But it’s a giant leap for the growing field of neuromorphic engineering—and one of the first, critical steps in creating a neural prosthesis to restore movement. Spinal cord injuries disrupt the brain-to-muscle cord communication— and subsequent motor control—but the local circuits in the spine are generally left intact below the lesion. “We’ve replicated some of the biological circuits on the chip to create the signals that generate locomotion by direct communication with muscles,” Etienne- Cummings explains. “In the future, the chip will actually communicate with spinal cord’s natural neural circuits that communicate with the muscles.”
He estimates that a neural prosthetic for spinal cord patients is about a decade away. “There’s still a lot of work to be done, both at the spinal cord physiology/anatomy and artificial controls of these systems,” he explains. “We are developing the next version, an implantable chip that will seamlessly interface with the nervous system to record directly from the spinal cord, process the information, and stimulate the cord directly.”
“We’re extracting essential [biological] components and implementing them to reproduce functionality,” says R. Jacob Vogelstein, a Hopkins PhD student in Biomedical Engineering, who works on prosthetics in Etienne-Cummings’ lab. “It’s a very small miracle, except we know how the miracle works because we built it.”